1. INTRODUCTION
Quantifying uncertainty in thermophysical properties of metals and alloys is a prerequisite for the manufacturing of reliable products and more importantly in development of new high performance alloys. Yet, rigorous uncertainty quantification is rarely found in most literature values reported for these properties. The present norm is to report mean and standard deviation of experimental values which fails to provide any insight on the underlying distribution of unknown residuals. Due to this poor variability reporting, the true extent of error propagation during uncertainty quantification are often overlooked—which hinders the true utilization of the significant potential of the quality of the metal additive manufacturing. Systematic reporting of uncertainty quantification and uncertainty management can lead to understanding of the variation in the quality of the manufactured parts.
A systematic facility performance evaluation (FPE) based on accuracy and precision developed by the author (Nawer & Matson 2023) was used to evaluate the reliability of the reported properties. Accuracy can be measured with respect to an accepted literature value and precision can be measured from uncertainties associated with each measurement. Uncertainty analysis was conducted using the widely accepted metrological International Standards Organization’s Guide to the Expression of Uncertainty Measurement (GUM) (JCGM/WG1 2008) rules. This FPE technique has successfully been utilized to evaluate performances of several levitation facilities for pure Zirconium (Nawer & Matson 2023), pure Gold (Nawer et al. 2023a) and Ni-based superalloy CMSX-4 Plus (Nawer et al. 2023b). This FPE technique was extended to evaluate accuracy and precision of density, volumetric thermal expansion coefficient (CTE), surface tension and viscosity measurements of another pure lmetal, Platinum.
Platinum has been widely used in various industrial and commercial applications due to its inert nature, high corrosion and oxidation resistance, high melting point coupled with good catalytic activity and biocompatibility (Knapton 1979). Apart from its vast usage in the chemical industry as a catalyst and numerous medical and jewelry applications, Platinum-based superalloys are used in high temperature space applications (Behrends et al. 2000). It has been extensively studied using several ground-based levitation facilities due to its excellent physical characteristics and chemical stability (Wilthan et al. 2004; Ishikawa et al. 2006; Paradis et al. 2014; Watanabe et al. 2020) which makes it a perfect candidate for microgravity experiments. Microgravity testing is used to provide enhanced fidelity (Egry 2004) of results purportedly due to three main effects. First, without strong gravitational accelerations and with reduced levitation forces a more spherical sample allows for better analysis of experimental behavior. Second, better control of convection in space results in higher measurement precision. Third, sedimentation and buoyancy induced segregation are eliminated in reduced gravity. These unique advantages of microgravity environment provide the researchers with an excellent opportunity to quantify FPE across various levitation platforms. Platinum was chosen as a baseline reference material for this study along with Gold and Zirconium as a part of Microgravity Physical Sciences Communities international collaborations on property measurements (Matson et al. 2016). A series of tests has been conducted using containerless electrostatic levitation (ESL) technique to study the uncertainties associated with thermophysical property measurements of this noble metal. This paper provides insights into the FPE of two space and ground-based ESL facilities with an aim to encourage appropriate reporting on uncertainties across the scientific communities.
2. DATA AND METHODS
Two ESL facilities were used for investigating thermophysical property of pure platinum (99.95%) Lot number 16470 from Surepure Chemetals Inc. for this study. Microgravity testing was conducted using Japanese Aerospace Agency’s Electrostatic Levitation Furnace (ELF) facility on the KIBO module of International Space Station (ISS) (Tamaru et al. 2018). Experiments were conducted from the command control station at JAXA Tsukuba Space Station in Tsukuba, Japan. In parallel, terrestrial experiments were conducted using the NASA Marshall Space Flight Center’s (MSFC) ESL facility in Alabama, USA (Nawer et al. 2020a). Both facilities use laser heating system, and both monitor sample surface temperature using infrared (IR) pyrometers. Sample shadow images were used for density and CTE measurement during free cooling of samples in both facilities. Platinum has higher work function, similar to gold (Sachtler et al. 1966), which made it quite difficult to remove electrons from the solid surface during heating and achieve a stable levitation during space testing. During the ground-testing under controlled slow heating conditions, droplet oscillation was successfully induced on the molten sample during several temperature holds. High-speed digital video recordings for these tests were used for surface tension and viscosity analysis. Fig. 1 shows typical images of the post-processed sample surfaces, one from each facility, obtained using scan electron microscopy (SEM) at the Institute of Materials Physics in Space German Aerospace Center (DLR), Cologne.
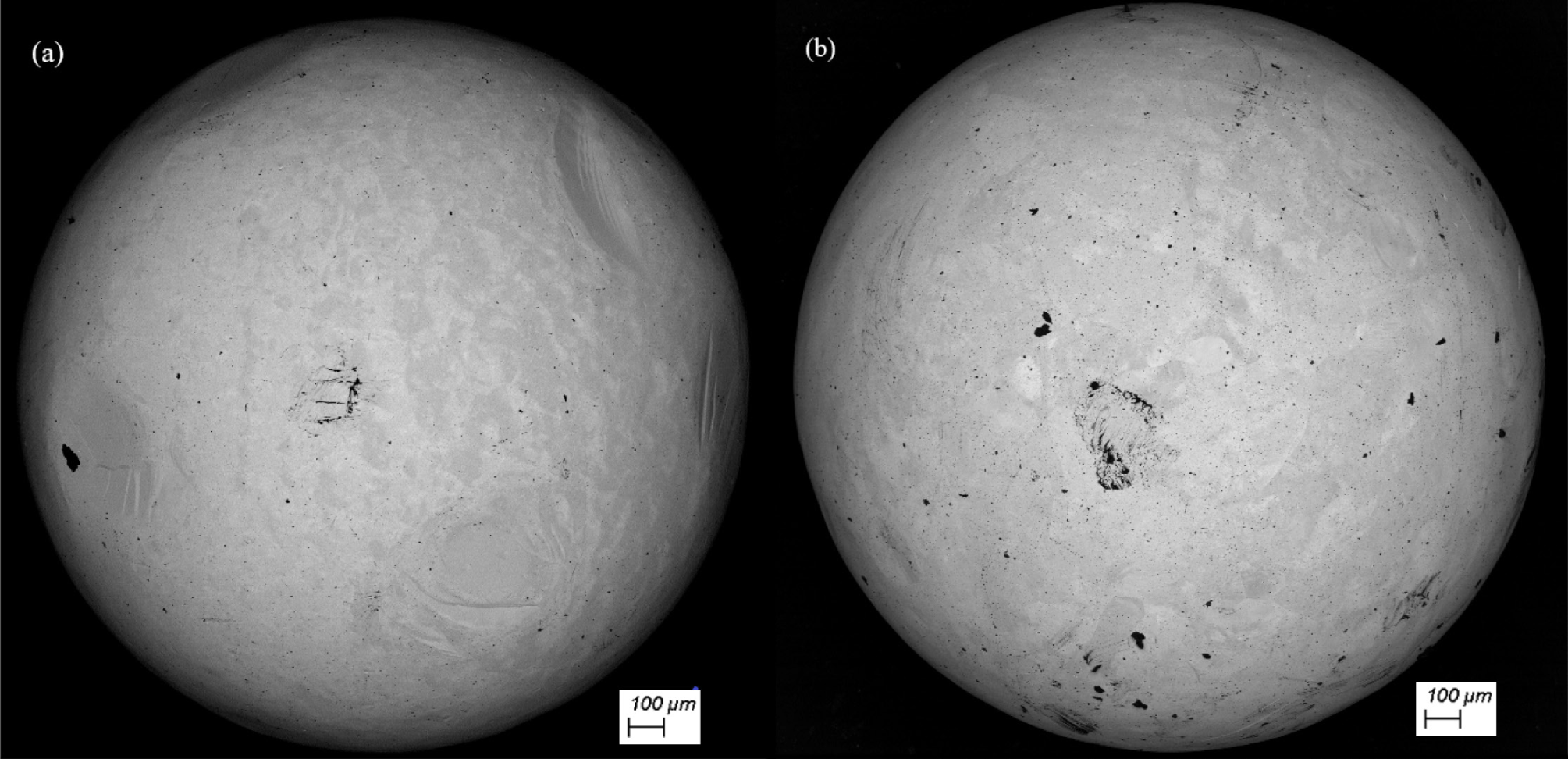
Evaluation of video data of the molten sample was performed using a customized edge detection algorithm with sub-pixel precision. The radius (r) of the near-spherical sample was first fitted with a 6th order Legendre polynomial function (Bradshaw et al. 2005) and then volume (V) was measured using vertical axis of symmetry as a function of polar angle (θ):
Dynamic sample mass was estimated after considering mass loss due to evaporation using Langmuir’s equation (Nawer et al. 2020b). Density of the liquid sample was then measured from the dynamic mass (m) and apparent volume (V). Volumetric CTE (β) at constant pressure can also be measured from the volume of the liquid at melting by considering the change in the slope during free cooling.
Droplet oscillation was induced on the molten sample by superimposing a sinusoidal voltage on the electrical field for a short duration (Cheng 1985) and then the sample oscillatory motion was allowed to dampen out by its viscosity once the excitation is removed. The natural frequency (fn) of the sample at mode l = 2,0 was identified by conducting frequency sweeps during Faraday Forcing (Douady 1990) at a steady thermal hold as shown in Fig. 2(a). Two methods were used to confirm the findings from the frequency sweeps. The first method is maximum amplitude (MA) method (Egry et al. 1995) where the largest sample deformation, δ = ΔA / 6A0 was observed with an increasing application of the forced frequency. The second method is the frequency crossover (FC) method (Nawer & Matson 2023) which as identifies the natural frequency at the intersection of the linear fits of forced and damped frequencies. From the frequency sweep shown in Fig. 2(a), the natural frequency identified by MA method is 154.39 Hz and FC method is 154.81 Hz. Both of these frequencies are in excellent agreement.
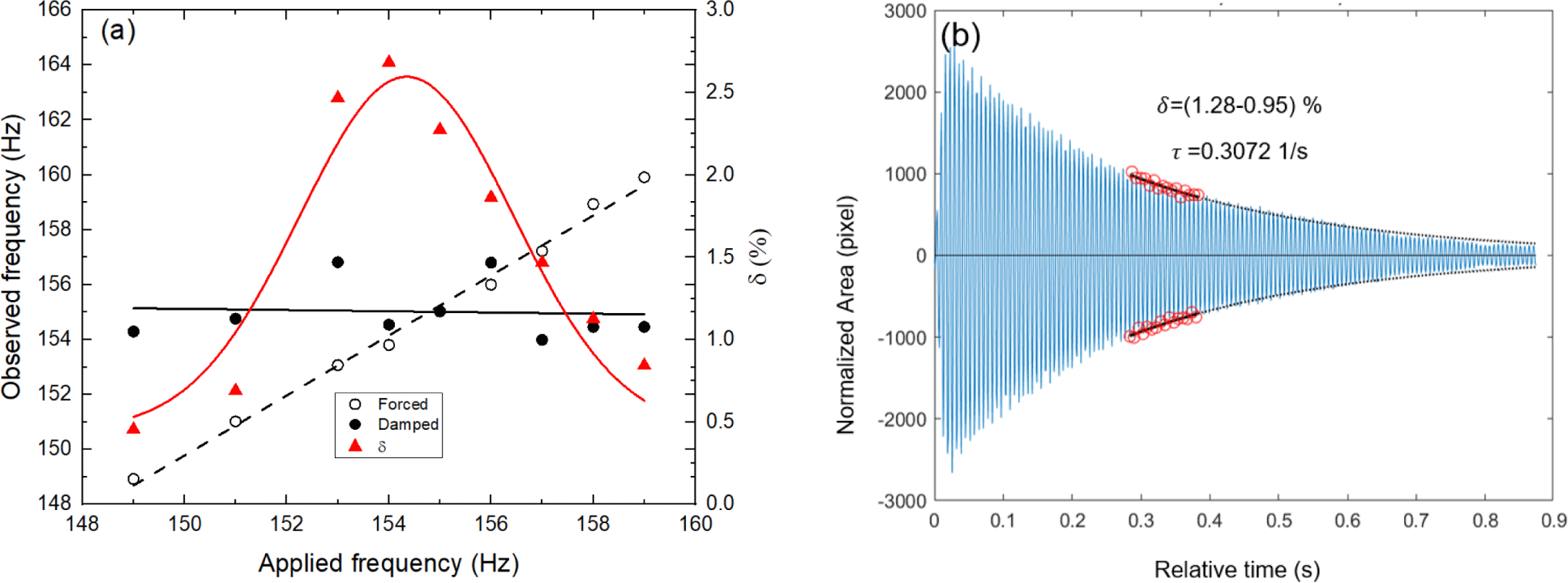
Surface tension (σ) of the liquid sample was measured by using the identified mode l = 2,0 frequencies. Viscosity (η) was measured from the sample density, unperturbed radius (r0) and time constant (τn) of the decaying signal when the forcing was ceased as shown in Fig. 2(b).
3. RESULTS AND DISCUSSION
Platinum was successful levitated and melted on both ground and space facilities. Density in the liquid and undercooled phase measured from these facilities are shown in Fig. 3 along with the reported literature values. Linear regressions conducted on the ISS-ELF dataset and all four datasets combined from NASA MSFC ESL facility are:
ISS-ELF:
NASA MSFC ESL:
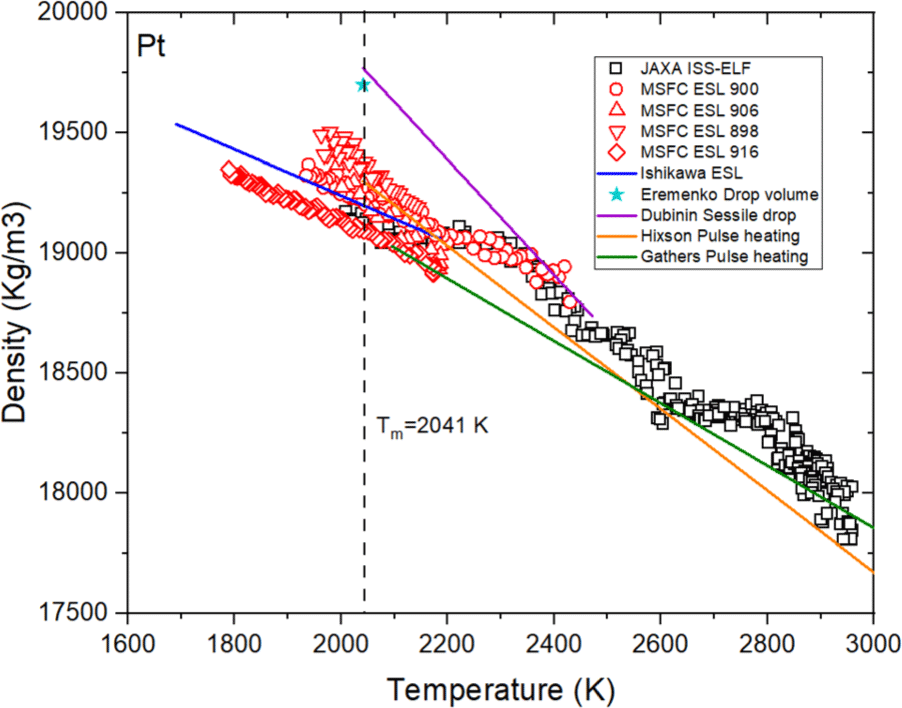
The measured density at the melting point from ISS-ELF is within 1% of the density measured at NASA MSFC ESL. Both values are within 1% of ground-based ESL values reported by Ishikawa et al. (2006) and both are also within 3% of sessile drop method values reported by Dubinin et al. (1975), and pulse heating methods by Hixson & Winkler (1993) and Gathers et al. (1978). The slope of the individual datasets from NASA MSFC ESL shows slight deviation which can be attributed to the accuracy of the pyrometer data. The density measured in the ISS-ELF shows a deviation from the expected linear behavior above a temperature 2,200 K which is induced by the noise from sample movement during the experimentation.
CTE for molten platinum as measured from ISS-ELF testing is (10.9 ± 0.5) × 10–5 K–1 which is twice the reported value by Ishikawa et al. (2006). The average measured value of CTE from MSFC ESL testing is (7.2 ± 0.2) × 10–5 K–1 which is 44% higher than the measured values by Ishikawa et al. (2006).
Droplet oscillation was successfully conducted at the NASA MSFC ESL facility. Surface tension was measured using natural frequencies identified by both FC and MA method as shown in Fig. 4(a). Unlike for other metallic melts (Nawer & Matson 2023), the linear trend of observed damped frequencies during the frequency sweep did not change significantly with the applied frequency as shown in Fig. 2(a). Thus, a third measurement approach known as the damped response (DR) method can be proposed for surface tension measurement as shown in Fig. 4(a). The apparent scatter seen in Fig. 2(a) indicates that the DR values are deemed to be of lower confidence compared to the higher confidence seen using FC method. Intercept regression error may be used to quantify systematic error introduced by a specific analysis technique, and the coefficient of variation, or the ratio of standard deviation to population mean was 11.8% and 12.55% for the DR and FC methods, respectively. The surface tension values are in good agreement with literature values as measured by Ishikawa et al. (2006) using ground-based ESL. Quantification of trends in surface tension were not conducted due to the small-sized dataset available in the current work.
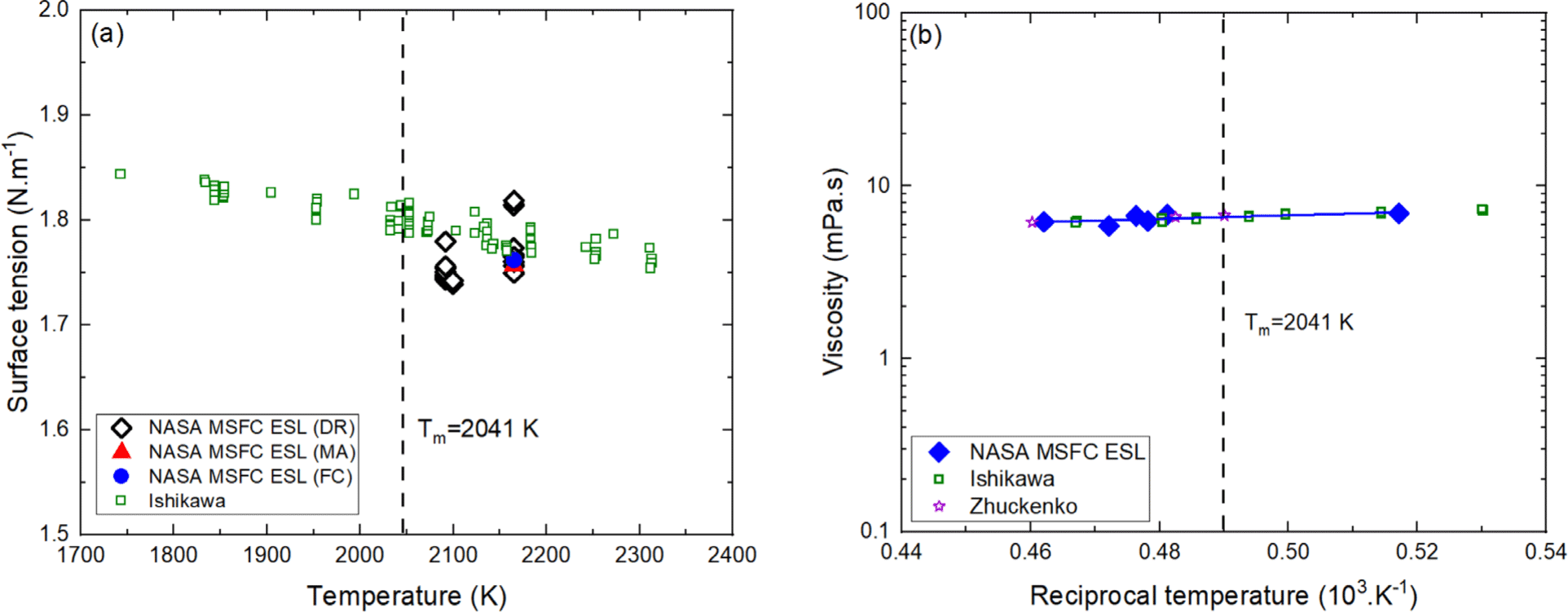
Viscosity of liquid platinum was measured at the NASA MSFC ESL and are shown in Fig. 4(b). The measured viscosity values are in excellent agreement with values reported by Zhuchenko et al. (1977) and Ishikawa et al. (2006).
A detailed uncertainty analysis was conducted on the measured properties from both ESL facilities maintaining the GUM guidelines and the results of this evaluation are listed on Table 1. Platinum samples processed on the ground exhibited less noise on the recorded temperature compared to the space samples because of higher application of electrostatic force resulting into and enhancement on the restriction of sample movement. Ground-tests were conducted in ultra high vacuum environment which resulted in higher evaporation compared to the space-tests where the samples were tested in a shielding Argon gas environment reducing mass loss. This contributed to higher uncertainty in mass for ground tests. ISS-ELF samples exhibited slightly higher uncertainty in sample radius and volume measurement obtained from the image analysis. Uncertainty in density was measured by considering both uncertainties from mass and volume measurements. The estimated uncertainty in the measured melting point density for ISS-ELF is 19,234.62 ± 294.84 kg·m–3 and for NASA MSFC ESL 19,196.34 ± 287.7 kg·m–3. Uncertainty in the measured CTE using ISS-ELF is estimated to be higher compared to the NASA MSFC ESL due to higher uncertainties in volume and slope. Uncertainty in surface tension was estimated by considering uncertainties from mass and frequencies. The estimated uncertainty in surface tension measured at the melting point (which was estimated from conducting a linear fit on the measured values) of 1.73 ± 0.02 N·m–1. Uncertainty in melting point viscosity is estimated to be 6.63 ± 0.22 mPa·s which was measured by considering uncertainties from radius, density, and time constant.
The reported uncertainties from Table 1 were used to calculate coefficient of variation which can be used to represent precision and deviation from the literature value can be used to represent accuracy:
Visualization of accuracy and precision for the measured properties can be demonstrated using a measurement of accuracy and precision (MAP) plot. Accuracy differs based on the use of literature value and previously reported literature values obtained using levitation were used for consistency. The smaller the deviation indicates the higher the accuracy is. The positive and negative shifts on the deviation also indicate if the measured properties are higher or lower compared to the literature values. Precision is estimated from the experimental values obtained from this study and are recommended for FPE. Similar to the accuracy, the lower values of coefficient of variation indicate higher measurement precision. Hence, lower values of deviation and coefficient of variation indicated higher accuracy and precision.
In Fig. 5, MAP plots of platinum for four measured properties at its melting point are shown along with the previously reported zirconium measured in ISS-ELF, ISS-electromagnetic levitation (EML) and NASA MSFC ESL (Nawer & Matson 2023). Accuracy of density and surface tension for platinum was displayed based on using Ishikawa’s ESL measurement (Ishikawa et al. 2006) as the reference literature value. Accuracy of CTE was displayed using Dubinin’s reported value of 0.000121 K–1 using Sessile drop method (Dubinin et al. 1975) as the reference value. Accuracy of viscosity was displayed using (Ishikawa et al. 2012) as the reference literature value.
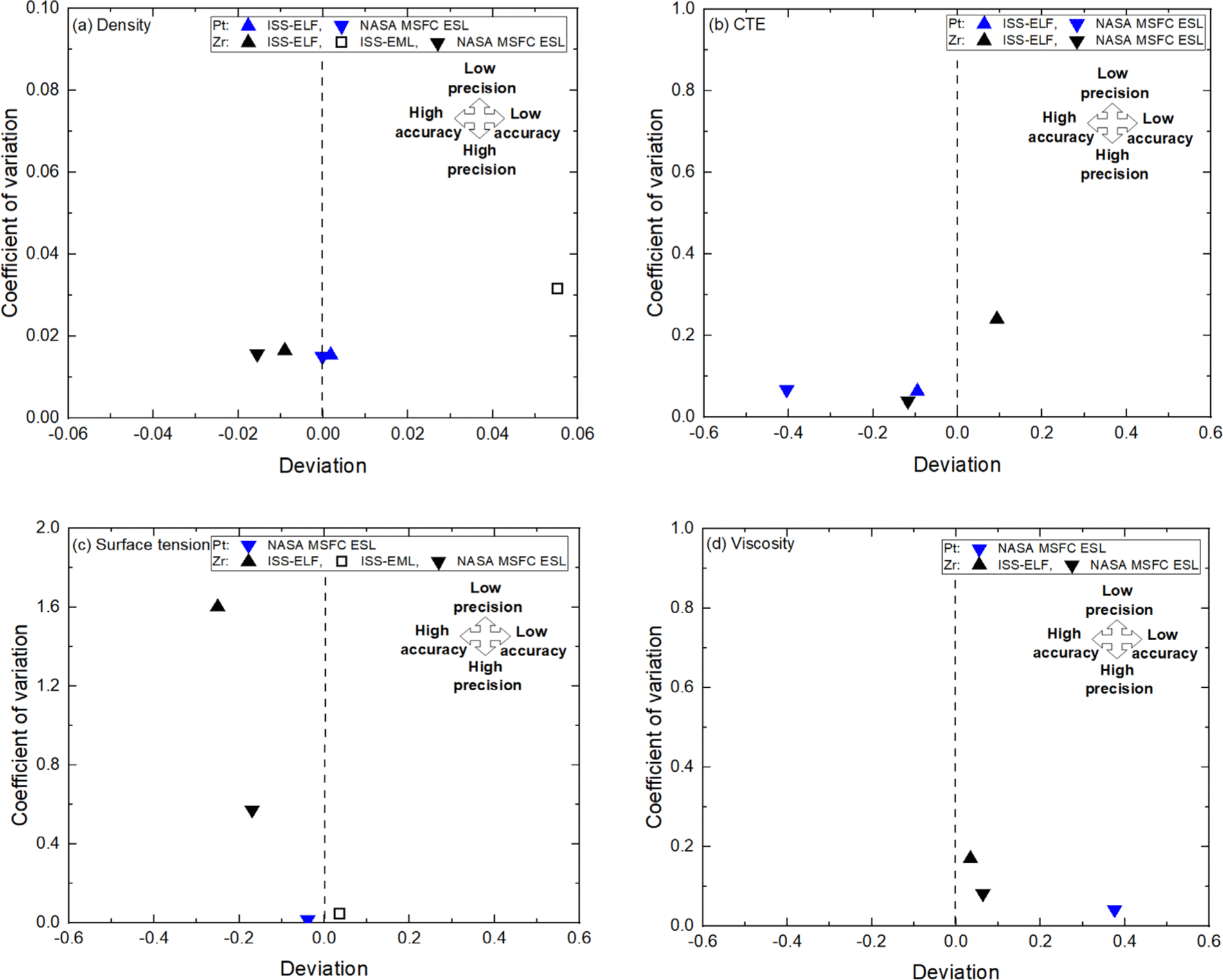
Density measurements from both facilities have similar accuracy and precision as shown in Fig. 5(a). These values have similar precision and better accuracy compared to the Zr values. CTE values measured in space has better accuracy and similar precision compared to the ground values as shown in Fig. 5(b). They also have similar precision compared to the ground based Zr tests. Accuracy and precision of surface tension measurements are shown in Fig. 5(c), the NASA MSFC ESL has higher accuracy and precision compared to the Zr tested in ground and space ESL; It also has similar precision compared to the Zr processed on using ISS-EML. Viscosity measured using the NASA MSFC ESL has higher precision, but lower accuracy compared to the Zr processed both in ground and space as shown in Fig. 5(d).
4. SUMMARY
Density, CTE, surface tension and viscosity of platinum in liquid and undercooled state were successfully studied using ISS-ELF and NASA MSFC ESL facilities. Density measured from on both facilities are in excellent agreement with reported literature values. CTE values measured from on both facilities are higher compared to the reported literature value. Surface tension and viscosity values are in good agreement with the reported literature values. The associated uncertainties with all four property measurements were reported using GUM guidelines. Both facility performances were evaluated numerically using the FPE benchmarking MAP plots. In future, this FPE would be extended to other levitation facilities to truly understand the effectiveness of the measurements from these two facilities. This helps researchers define which classes of materials are best measured in a specific facility since each has unique individual strengths and weaknesses.