1. INTRODUCTION
Muons and neutrons are produced by interactions between primary cosmic ray particles and the nuclei of atmospheric gas compounds. They can then be detected on the ground or underground. Interactions with the atmosphere are important factors determining the intensity of muons and neutrons. The flux of neutrons as the secondary cosmic ray particles can be modulated by solar activity and solar eruptions. They also experience diurnal variations by the local anisotropy of cosmic ray intensity during the Earth’s rotation (Oh & Yi 2006;Oh et al. 2010;Park et al. 2018). The solar cyclic variations in cosmic ray particles show an anti-correlation with the sunspot numbers as the solar activity. The trend of the neutron monitor data reflected the extremely weakened solar activity in the solar minimum between solar cycles 23 and 24 (Bieber et al. 2013;Oh & Kim 2013;Oh et al. 2013). Solar eruptions, such as coronal mass ejections and solar flares, can be projected to Forbush decreases of decreasing events, or ground level enhancements of increasing events (Forbush 1937;Forbush 1946;Oh et al. 2008;Oh et al. 2012).
In particular, muons that are generated from high-energy cosmic ray particles can be modulated easily by meteorological effects, such as temperature effect, and they show seasonal variations (Oh & Kang 2013). Seasonal variations in the muon intensity are caused by temperature and barometric effects that are associated with changes in the height and density of the atmosphere in summer and winter. The temperature effect can influence the generation of muons in the upper atmosphere, while the barometric effect can determine the survival of muons in the atmosphere (Agafonova et al. 2017).
The temperature effect on muons can be attributed to competition between pion and muon decay and their interactions with the nuclei of the air. Upon expansion of the atmosphere by heating, muons can experience either a negative temperature effect of a decrease through absorption. Higher temperatures reduce atmospheric pion absorption, which introduces a higher rate of muon generation as the positive temperature effect. Muons with typical energies for the ground detectors experience a negative temperature effect, while muons in the energies registered by underground detectors have a positive temperature effect (Berkova et al. 2011). For example, the increase in temperature between the layers where muons are produced (100 hPa) and the one where they interact (200 hPa) leads to a lower atmospheric density and a larger fraction decaying into muons (Bouchta et al. 1999). Bouchta et al. (1999) measured the variation of the muon flux with an AMANDA underground detector at the South Pole. They revealed a 10% effect in the positive correlation between the muon flux variation and the atmospheric changes. In addition, the ICECUBE collaboration (2011) found a strong correlation of the daily observed muon rate with the stratospheric temperature along with a ±8% annual modulation using 150 billion cosmic ray-induced muon events collected over four years by ICECUBE.
On the other hand, the temperature effect of a neutron monitor is caused by the involvement of unstable particles (muon, pion) in the generation of neutrons in the Pb absorber of an instrument (Dorman 1972). Fast particles produce neutrons in their nuclear interactions, while the stopped negative muons and pions generate them through the production and decay of mesoatoms (Dorman et al. (1990). Dorman et al. (1990) computed the temperature coefficients for five pairs of neutron monitor stations of observed seasonal waves. They then suggested that the observed seasonal waves contained the temperature effect. Belov et al. (1995) determined the temperature effect in the observed neutron monitored monthly count rates using the data of the worldwide network of neutron monitors and the atmospheric temperatures measured at the standard isobar levels during the 1957–1968 and 1979–1980 periods. They suggested that the season wave is caused by the atmospheric temperature dependence and the north-south anisotropy.
Agafonova et al. (2011) reported a 1.5% periodic variation in muon intensity with a period of one year using a Large Volume Detector located at the Gran Sasso underground laboratory. On average, the maximum muon intensity corresponds to July, and the minimum occurs in January. They also examined the variations of neutrons produced by muons in a scintillator, and their variations are in phase with variations of muons, coincident with temperature. Agafonova et al. (2017) measured the seasonal variation of neutrons induced by muons and reported a six-fold higher amplitude to muons.
In previous studies, the seasonal variations on muons are well defined by the temperature effect. On the other hand, it is not sufficient to explain the seasonal variations in the atmospheric and muon-induced neutrons. Although it has been explained, it is only by meteorological effects not by the intrinsic origin of the cosmic ray particles from space. Therefore, this paper examined the specific position, where more cosmic ray particles enter the orbit of revolution. If the seasonal variations of the cosmic ray intensity result from extraterrestrial factors, such as the position of the Earth in space, the sources of cosmic ray particles from dynamic celestial activity, such as the explosion of a supernova and active nuclei, can be found. Various modulations of neutrons can be observed. This paper discusses the statistical method to eliminate solar cyclic variations and temporary variations by solar eruptions effectively.
2. DATA AND METHOD
The Oulu neutron monitor has been collecting the cosmic ray intensity data since it started operation in 1964. The Oulu station also has been providing researchers with collected data on different time scales, i.e., hourly, daily, monthly, and yearly data. From the Oulu station, the daily mean data were downloaded and analyzed to remove the diurnal variation of the data. Before 1974, the detector was located in a building with a flat roof where snow could accumulate during winter (Tanskanen 1968). Usoskin et al. (2017) reported that strong seasonal peaks were caused by snow on the roof during the winter months before 1974. The Oulu neutron monitor was finally settled in a building with a pyramid-shaped warmed roof, so that snow could not accumulate above the neutron monitor since 1974. Therefore, the Oulu neutron monitor experienced very strong seasonal variations due to snow on the roof for the period 1964–1973. Because this meteorological effect can influence the results of the paper significantly, this period was excluded from seasonal variation analysis. This study did not exclude the cosmic ray variations by solar effects, such as Forbush decreases and ground level enhancements, which are the issues to be discussed in the last section. The main goal of the paper was to eliminate the effect of solar activity across time. To that end, two different transformations were first considered: transformation with respect to (w.r.t) the grand mean and transformation w.r.t the yearly mean. More specifically, the transformation w.r.t grand mean is defined as follows:
where xji is cosmic ray intensity of day j of year i, i = 1974, ⋅⋅⋅, 2019, j =1, ⋅⋅⋅, 365, and x, and x is the grand mean of all data. Similarly, transformation w.r.t the yearly mean is defined as
Although the distribution of cosmic rays is assumed to be isotropic, cosmic ray particles experience different solar activity, which varies with the sunspot numbers. This is because of the magnitude of the solar magnetic field, which displays the solar cyclic variation of the cosmic ray intensity at the Oulu neutron monitor, as shown in Fig. 1. Fig. 1 shows the monthly mean data from April 1964 to December 2019.
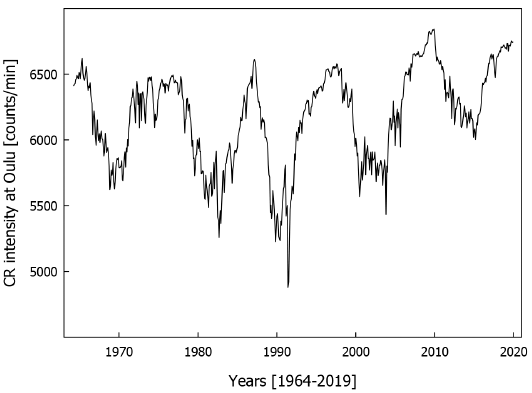
The cosmic ray intensity is a function of time in the phase of the solar cycle. This trend is especially apparent in Fig. 2, which shows the time variation of the daily cosmic ray intensity on the days of the vernal and autumnal equinoxes and summer and winter solstices from 1964 to 2019. The Earth sets the same position on each day of these four days every year. On the other hand, the profile of cosmic ray intensities on the same day for 56 years shows the solar cyclic variations. The four days of interest are represented in different colors: spring (in green), summer (in red), autumn (in blue), and winter (in black). Although the Oulu neutron monitor experienced seasonal variations due to the accumulation of snow, the cosmic ray intensity followed the solar cyclic variations in the early period before 1974.
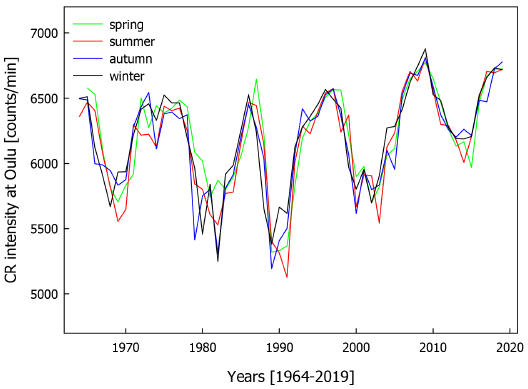
3. RESULTS
As mentioned in Section 2.1, the cosmic ray intensities during January 1974–December 2019 were transformed in two different ways: (a) transformed w.r.t. the grand mean and (b) transformed w.r.t the yearly mean. After the transformation, data were collected on the same day. Each day consisted of approximately 46 observations, and the mean or median was calculated. Fig. 3 shows the mean-based (in black) and median-based (in pink) profiles. The thick black and red lines indicate the smoothed mean-based and median- based profiles. The data for February 29 every four years were removed for the consistency of statistical analysis.
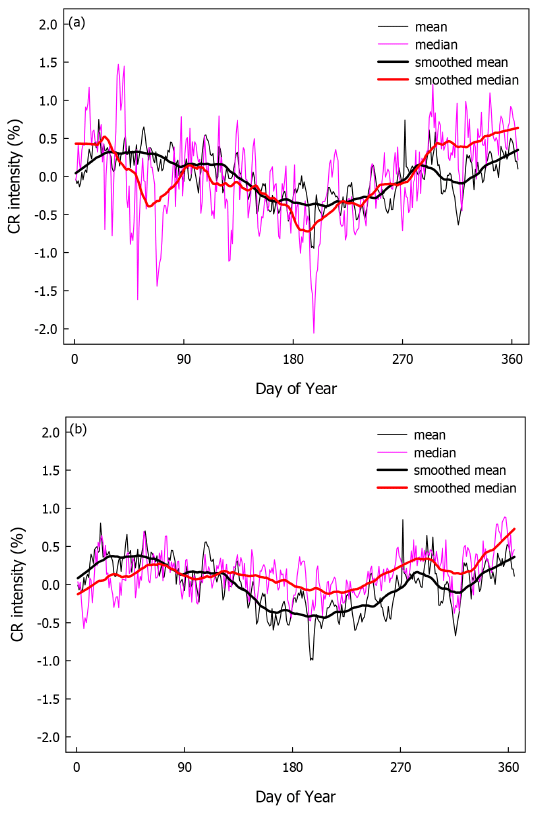
Fig. 3 presents the cyclic variations from the mean-based and median-based profiles. The Cox and Stuart trend test was used to examine whether there are time-dependent variations (seasonal trend). The null hypothesis was that there is no time-dependency, i.e., independent observations. To test the null hypothesis, the Cox and Stuart trend test was applied to the smoothed profiles in Fig. 3, which is summarized in Table 1. The table includes the p-values for the four smoothed profiles. The data were not independent because all p-values were too small (usually, the significance level of 0.05 is used). In other words, there was some seasonal trend.
Profile | w.r.t the grand mean | w.r.t the yearly mean | ||
---|---|---|---|---|
|
|
|||
Mean | Median | Mean | Median | |
p-value | 5.464 × 10–11 | 0.0001241 | 5.464 × 10–11 | 1.813 × 10–10 |
The mean-based profile was different from the median- based profile in spring (Fig. 3a). In late autumn, however, two profiles were close to each other. In contrast, two profiles (mean-based and median-based) in Fig. 3b show a more similar pattern across time. In addition, the meanbased and median-based profiles in Fig. 3b revealed clear seasonal variations. If the seasonal variation comes from extraterrestrial effects, cosmic ray particles enter more in the early spring and early winter. If this is not, and it is caused by terrestrial effects, such as temperature or barometric effects, the seasonal variations would show a reduced flux during summer, as shown in the muon intensity.
Fig. 4 shows the difference between the mean-based and median-based profiles for each normalization: w.r.t the grand mean (in black) and w.r.t the yearly mean (in pink). The jagged line in black, corresponding to transformation w.r.t the grand mean in Fig. 3a, has violent fluctuations with respect to the horizontal reference line. In essence, the ranges of the difference were 3.26 for the transformation w.r.t the grand mean (in black) and 1.50 for the transformation w.r.t the yearly mean (in pink). In other words, the values for the case of the grand mean are more widely distributed. To examine the statistical variability for two different transformations, the null hypothesis of equal variance was tested:
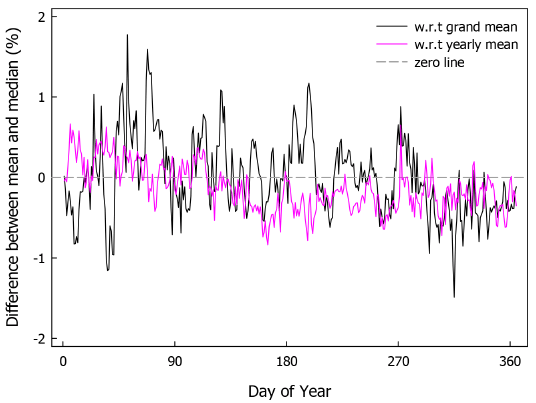
where is the variance of the difference between the mean and median for the grand mean case, and is that for the yearly case. The test revealed p-values less than 2.2 × 10–16, suggesting that the null hypothesis was rejected. As a reference, the variance estimates for the difference between the mean-based and median-based profiles are provided: normalized w.r.t. the grand mean and w.r.t. the yearly mean (Table 2). As listed in the table, the grand mean case has much larger (i.e., three times) variance than the yearly mean case. From a statistical point of view, the difference between the mean and median in Fig. 3b is relatively moderate, and it looks more statistically stable.
w.r.t the grand mean in Fig. 3a | w.r.t the yearly mean in Fig. 3b | |
---|---|---|
Variance estimate | 0.24 | 0.08 |
4. SUMMARY AND DISCUSSION
In contrast to previous studies that examined the season- al variations due to terrestrial factors, such as the temperature effect, this study examined the seasonal variation by extraterrestrial factors, such as the position of the Earth in the orbit of revolution. This study examined the daily data at the Oulu neutron monitor from January 1974 to December 2019. To eliminate the solar cyclic variations, the profile of cosmic ray intensity for 365 days was calculated using two different transformations w.r.t the grand mean and w.r.t the yearly mean.
Fig. 3 and Table 1 show the seasonal variations of the cosmic ray intensity at the Oulu neutron monitor with a magnitude of approximately 1%. The data after transformation w.r.t the yearly mean show more statistical stability and apparent seasonal variations that the cosmic ray particles enter, as shown in Fig. 4 and Table 2. In particular, the more cosmic ray particles come in early spring and early winter and less in summer. More particles in early spring and early winter enter due to the position of the Earth in its orbit of revolution, and particles are reduced in the summer because of the meteorological effect. The seasonal variation in Fig. 3b appears consistent with the terrestrial origin. For example, fewer counts are observed during the warm season (summer) when the atmosphere is thermally expanded. The decreasing trend in the summer is similar to variations of ground muon intensity by de Mendonça et al. (2016). Their results suggested that a decrease in ground muon intensity occurs during summer in the high-latitude regions by analyzing the temperature effect on the Global Muon Detector Network. The seasonal variations at the Oulu neutron monitor may result from the seasonal variations of muon intensity affected by the atmospheric density and temperature. On the other hand, muons are generated by high-energy protons, but the neutrons detected at the Oulu neutron monitors are mostly generated from low-energy protons due to low cutoff. Therefore, this study could not determine that the seasonal variations at the Oulu neutron monitor are due to meteorological effects. More studies will be needed to analyze more neutron monitors located at different latitudes and meteorological factors.
A future study will investigate the development of a statistically reliable process, which eliminates the effect of solar activity and temporal variations by solar eruptions. From this, the seasonal variations of the cosmic ray intensity by extraterrestrial factors can be determined.