1 INTRODUCTION
The announcement of the 2nd Basic Plan for Promotion of Space Development in December 2011 marked the beginning of the first Korean planetary exploration. Through this plan, the Moon was selected as the first target destination. However, Korea started full-scale space development with the Space Development medium and long-term Plan (1996- 2015) in April 1996. At that time, bulk of the development plan focused on the development of earth-orbiting satellites and space launch vehicles. In May 2005, the Korean Space Development Promotion Act was promulgated “to facilitate the peaceful use and scientific exploration of outer space and to contribute to national security, the sound growth of the national economy, and the betterment of citizens’ lives by systematically promoting the development of outer space and by efficiently using and managing space objects” (KLRI 2018). This act stipulated the establishment of the National Space Committee and the formulating of basic plans for the promotion of space development every five years. In June 2007, the 1st Basic Plan for Promotion of Space Development was announced. Through this plan, the necessities of planetary exploration were declared with emphasis on the technological readiness to prepare for future planetary missions. Additional details of Korean planetary mission plans were declared in the 2nd Basic Plan for Promotion of Space Development in December 2011. In November 2013, Korea announced the Space Devel-opment medium and long-term Plan (2014-2040) that was a modified and updated version of the 2nd Basic Plan for Promotion of Space Development. In this plan, a wide range of future Korean planetary exploration plans was declared, including the moon, Mars and asteroid exploration. In 2013, the Korean Lunar Exploration Program (KLEP) was announced. The primary objectives of KLEP were to obtain knowledge of lunar science, develop innovative space technologies, investigate lunar resources for future utilization, and enhance national branding and public interest in further space exploration (Ju et al. 2013). To achieve these goals, the KLEP comprises two phases. In the first phase, the Korea Pathfinder Lunar Orbiter (KPLO) will be launched in late 2020 via an international collaboration. In addition, as part of the first stage, the Korea Deep Space Antenna (KDSA) will be constructed and operated to support not only KPLO missions, but also upcoming Korean planetary exploration missions. In the second stage, a lunar lander is planned to be developed by Korea, as well as being launched using a Korean launch vehicle. More recently, the 3rd Basic Plan for Promotion of Space Development was announced, in which it was declared that mission analysis and technical standard reviews for the moon-landing mission will take place from 2019. In addition, further plans for asteroid landings and sample return missions will be conducted by the end of 2035.
Certainly, the first step in realizing planetary missions is the associated trajectory design and analysis. Unlike earth orbiting missions, planetary missions are more demanding of well-rounded technological capabilities, specifically requiring an in-house capability to design reference trajectories and navigating technique to follow the designed trajectories. This is because securing the associated technology is critical from the point of view of not only system design but also the operation of planetary missions. On the basis of the designed reference trajectory, detailed mission design could be performed including generating requirements for spacecraft bus systems as well as ground systems. In addition, more in-depth and precise knowledge of deep-space navigation will allow more accurate target transfer trajectories and mission orbits around the planet, which will ultimately lead to more effective and safe operation of deep-space spacecraft. To date, the Korean astronautical society has conducted extensive trajectory design and analysis, not only from the mission design point of view, but also from an operational point of view. Wide ranges of studies, focusing on planetary missions, have been conducted by the Korean astronautical society. Among the studies conducted to prepare not only the KPLO mission but also future planetary missions, current work focuses firstly on the status of previous and current studies in Korea, specifically on flight dynamics and navigation for planetary missions. Further expeditions after completion of the KPLO mission, as well as efforts to prepare for future planetary missions conducted to date, are discussed. This study is organized as follows. In Section 2, previous studies devoted to secure the basic technologies for trajectory design for planetary missions in the early 2000s is discussed. Section 3 describes the current status right from the beginning when the KPLO mission funding started. The status of flight dynamics system (FDS) development as well as its verification and validation strategies is discussed in Section 3. In Section 4, expectations from the success of the KPLO mission and associated contributions to future planned planetary missions are discussed. A brief summary of past efforts by the Korean astronautical society to prepare future planetary mission is also discussed in Section 4. Concluding remarks are presented in Section 5.
2 PAST EFFORTS: STARTING WITH MARS MISSION TRAJECTORY DESIGN
The first trials to secure the basic technologies on trajectory design for planetary missions were attempted in early 2000s. Trajectory design for the fictitious Mars mission, as opposed to a lunar mission, was first attempted. Earth to Mars mission opportunities from the Naro Space Center were investigated, including the launch window as well as daily launch window analyses. A number of porkchop diagrams of launching in 2027 were studied, together with daily launch window comparisons between Naro and the Kennedy Space Center (Yoo et al. 2003). In addition to launch opportunity analyses of ballistic missions to Mars, Song et al. (2004a) extended launch window analysis capabilities using gravity assist techniques. Diverse launch options were investigated using single or multiple gravity assist options to Jupiter (Song et al. 2004a). Basic studies on deep space network (DSN) measurement modeling were also conducted to prepare navigation for future interplanetary missions (Kim et al. 2004). Kim et al. (2004) modeled fictitious DSN observables such as range, Doppler, and angular data with modeling of associated observational errors. The results were compared with Jet Propulsion Laboratory (JPL) results for validation. Orbit propagation software was developed (Song et al. 2004b) to prepare proximity operations around Mars. To ensure the fidelity of the software, the Mars50c gravity field and the Mars-GRAM 2001 models were used to account for non-sphericity and atmospheric drag effects of Mars. Software verification was also performed and the results indicated differences smaller than meter level in one Martian day propagation when compared to the Analytical Graphics Inc. (AGI) System Tool Kits (STK) results at that time (Song et al. 2004b). Song et al. (2005) conducted additional in-depth Mars transfer trajectory design and analysis using optimization techniques and establishing a trajectory correction maneuver (TCM) strategy by adapting the B-plane targeting method (Song et al. 2005). Detailed dynamic model development for interplanetary navigation purposes was also conducted by Park et al. (2005). The Cowell method for special perturbation theories was employed to develop detailed dynamic models with the inclusion of the relativistic effect of the Sun (Park et al. 2005). Entire phases of Mars mission, from Earth departure to Mars capture and mission orbit insertion were analyzed under the assumption of using the Korea Space Launch Vehicle (KSLV) (Song et al. 2006). In a Song et al. (2006) study, Naro Space Center was selected as a launch site, and relevant maneuver characteristics to perform Mars missions were analyzed, including trans-Mars injection, TCM, Mars orbit insertion, and orbit trim maneuver (OTM). A mass budget analysis was also conducted to estimate possible dry and fuel masses of the spacecraft with consideration of the KSLV delivery performance.
After partial completion of securing the basic technologies on Mars mission trajectory design and analysis, the Korean astronautical society changed the target to the Moon. On the basis of the Mars mission trajectory design and analysis previously conducted, preliminary design studies were initiated and performed to prepare future lunar missions. Song et al. (2008) developed preliminary lunar mission design software. The developed software utilized impulsive burn to design an Earth–Moon transfer trajectory and covered the Earth departure to lunar orbit acquisition (LOA) phases with consideration of the final mission operation orbit. In addition, functions of approximately estimating the fuel budgets of the spacecraft, ground contact schedule, and the angle geometry between Earth– Moon–spacecraft–Sun were included. Song et al. (2009a) later extended a previous study by applying a number of Earth–Moon transfer options such as using phasing loop strategy, as Song et al. (2008) only considered direct transfer options for Earth–Moon transfer. In 2010, Woo et al. (2010) performed optimal Earth–Moon transfer trajectory analysis with consideration of spacecraft visibility from the Daejeon ground station. This study was performed to partially consider operational constraints; successful execution of the trans-lunar injection (TLI) and lunar orbit insertion (LOI) maneuvers are critical factors among the various lunar mission parameters, and therefore, necessary to design an optimal lunar transfer trajectory that guarantees visibility from a specified ground station while executing these maneuvers. To prepare proximity operations around the Moon, Song et al. (2010a) developed a precise lunar orbit propagator. In the developed propagator, accelerations because of the non-spherical gravity of the Moon using the LP165P model, the point masses of the Earth, Moon, Sun, Mars, and Jupiter, as well as radiation pressures were considered. The lifetime of a lunar orbiter, time to hit a lunar surface without any station-keeping strategy, was also analyzed. Song et al. (2010a) reported that the lifetime of a lunar polar orbiter is significantly affected by the different degrees and orders of the lunar gravity model, by gravitational attractions of a third body (specifically the Earth), and by the different orbital inclinations. This software was later updated by Song & Kim (2015) by applying a well-known recursion formula that directly uses fully normalized associated Legendre functions to compute the acceleration because of the non-sphericity of the moon. By implementing this formula, a significant amount of computation time was saved, even with high-degree-andorder harmonic coefficients, and accuracy degradation that can arise during numerical simulations, was prevented (Song & Kim 2015). To establish more realistic design concepts, the TLI maneuver was optimized and analyzed by applying a finite thrust model (Song et al. 2010b). An optimal Earth– Moon transfer solution with finite thrust was derived and compared to those designed with impulsive thrust in previous studies (Song et al. 2010b). In addition to the TLI maneuver design and analysis with the finite thrust concept, Song et al. (2011) extended their previous study to include LOI burns. Song et al. (2011) analyzed the effect of finite burn delta-V losses during the lunar capture sequence, and also approximately estimated loss rates by comparing the values with those derived with impulsive thrust. Song et al. (2010b) and Song et al. (2011) recommended that careful consideration should be given to the estimation of mission fuel budgets, specifically if the preliminary mission studies assumed the use of impulsive thrust. Choi et al. (2013) designed and analyzed lunar orbiter trajectory options using a direct transfer trajectory. Song et al. (2008) had already analyzed Earth–Moon transfer characteristics using direct transfer options, and Choi et al. (2013) investigated the launch window including launch azimuth, delta-V profile according to TLI, and LOI burn positions. Choi et al. (2017) performed trajectory optimization using the pattern search method to minimize the delta-V magnitude required for Earth–Moon direct transfer. Song et al. (2013) analyzed the ground contact opportunity for the fictitious low lunar orbiter to prepare for a future Korean lunar orbiter mission. In the analysis of Song et al. (2013), four KOMPSAT ground stations are assumed to be the Korean future near earth networks to support lunar missions, and worldwide separated DSNs are also included during the contact availability analysis. Similar analyses were conducted by Song et al. (2014a) that focused more on lunar transfer and capture phases. Under the established conceptual future mission scenarios, detailed tracking schedules and expected measurement characteristics during critical maneuvers (TLI as well as LOI), specifically for the Deajeon station, were successfully analyzed (Song et al. 2014a). In the nominal lunar mission orbit, the orbiter will periodically execute maneuvers to maintain the lunar altitude boundary. A number of preliminary station-keeping strategies have also been investigated (Bae et al. 2013a, 2013b; Bae & Ju 2014). Although numerous preliminary flight dynamics studies on lunar missions have been performed, they have all used a trajectory design analysis approach. However, the development of orbit determination (OD) software and preparing successful navigation is another critical field that must be analyzed and studied in depth. A brief but essential development strategy for the lunar OD system was reported by Song et al. (2014b). Technical models of foreign agencies of lunar OD systems, tracking networks to measure the orbit, and collaborative efforts to verify system performance were reviewed. The preliminary development strategy for the Korean future lunar OD system was discussed with regard to the core technical issues of dynamic modeling, numerical integration, measurement modeling, estimation method, measurement system, as well as appropriate data formatting for interoperability among foreign agencies (Song et al. 2014b). For precise computation of the shadow events of a lunar spacecraft caused by the Earth's shadow, Song & Kim (2016) analyzed the effect of the Earth's oblateness on predicting the shadow events, and highlighted the importance of modeling the oblate shape of the Earth when predicting the shadow events of a distant lunar spacecraft.
3 RECENT STATUS: KPLO MISSION TO MOON
The funding of the first Korean lunar orbiter, the KPLO, was initiated in 2016 as part of KLEP announced in 2013. In 2016, The Korea Aerospace Research Institute (KARI) and National Aeronautics and Space Administration (NASA) agreed on the details and role assignments for cooperation regarding lunar exploration technology. The KPLO will make provision for a NASA payload and KARI will receive technical consultation from NASA including deep-space navigation, and deep-space network antenna establishment. To ensure the successful launch of the KPLO, the KARI is now conducting extensive trajectory design and analysis activities, including joint efforts between KARI and NASA to validate the trajectory design and navigation performance.
The KPLO is expected to be launched in late 2020, with the nominal mission orbit around the Moon at an altitude of approximately 100 ± 30 km. The mission duration will be no longer than 12 months, including the commissioning phase. The launch mass of the KPLO is expected to be approximately 550 kg (with the scientific payload mass of about 40 kg) and will be launched by the private US aerospace manufacturer, Space-X. Four 30 N thrusters will be used as the primary thrusters for large burns, such as trajectory corrections during the transfer and the LOA phase. Four 5 N thrusters will be used primarily for small burns, such as momentum dumping and orbital maintenance maneuvers during the nominal mission phase. For the KPLO science mission, a total of five instruments will be onboard to complete the primary mission objectives, including verification of the disruption tolerant network (DTN) methodologies. The KPLO instruments and developers are as follows: LUnar Terrain Imager (LUTI) by KARI, polarimetric camera (PolCam) by the Korea Astronomy and Space Science Institute, KPLO Gamma Ray Spectrometer by the Korea Institute of Geoscience and Mineral Resources, KPLO Magnetometer by KyungHee University, and finally, a shadow camera (ShadowCam) by researchers at Arizona State University and Malin Space Science Systems as a NASA contribution. In addition, the Electronics and Telecommunications Research Institute of Korea and NASA are working together to develop and demonstrate DTN technologies. (Song et al. 2017a). Fig. 1 shows the current configuration of the KPLO spacecraft in-flight operation.
After numerous tradeoff studies between direct transfer, phasing loop transfer, and weak stability boundary transfer Earth–Moon transfer options, the phasing loop was selected as a baseline for KPLO transfer options. In addition to the selection of the phasing loop transfer option, benefits and drawbacks of different numbers of phasing orbits (1.5, 2.5, and 3.5 phasing orbits) were also critically revisited, and 3.5 phasing loop orbits were selected as a baseline for KPLO transfer options. A significant benefit of the selection of 3.5 phasing loop orbits is a lower sensitivity to initial launcher errors compared with direct transfer. Therefore, by selecting the 3.5 phasing loop orbit, the required amount of TCM, which typically increases significantly after 24 h of TLI burn in cases where the direct transfer option is selected, can be greatly reduced. In addition, the 3.5 phasing loop can guarantee similar lunar arrival times and geometries by adjusting the final phasing loops, and additional time slots for system and operation checkouts can also be secured. As the reference trajectory of KPLO was selected as a 3.5 phasing transfer, Choi et al. (2016) analyzed a visibility condition of KPLO based on the combination of various candidate ground stations and the masking angles by utilizing 3.5 phasing options. In addition, the optimum satellite–Earth–Moon (SEM) angle is suggested in Choi et al. (2018a) from a number of viewpoints including launch epoch, coast duration, perigee altitude, and delta- Vs, regarding not only the transfer but also LOA phases as the SEM angle defined in the Earth–Moon rotating frame is a critical constraint in evaluating the 3.5 phasing loop transfer trajectory. Choi et al. (2018b) recently conducted a number of trade-off studies of KPLO transfer options to minimize the delta-V, including phasing loop options, translunar inclinations, and 1st apogee altitude. Lee et al. (2017a) examined the effect of steering angle constraint during finite burn maneuvers to optimize fuel usage during a finite burn. Figs. 2 and 3 show 3.5 phasing loop transfer trajectory in an Earth-centered inertial frame and an Earth–Moon rotating frame, respectively. For effective station-keeping, Park et al. (2018) recently proposed an enhanced control technique to reduce delta-V costs for the KPLO mission and demonstrated it by comparing it to algorithms implemented in previous lunar reconnaissance orbiter (LRO) and KAGUYA missions.
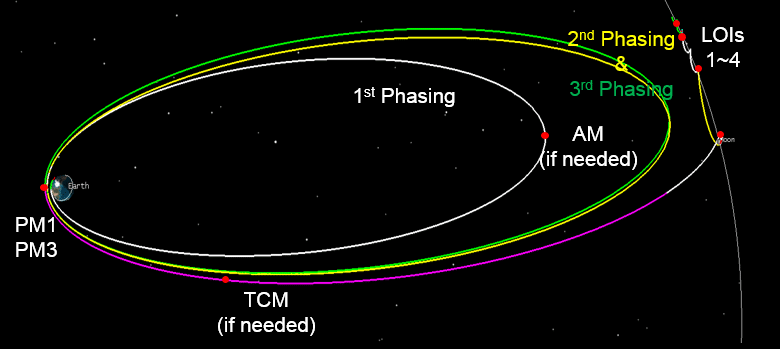
Various error analyses on maneuver execution were also conducted. Analysis of the phasing-loop transfer trajectory according to the position uncertainty of the KPLO, including initial dispersion analysis as well as midcourse trajectory correction maneuvers, were conducted (Bae et al. 2016a, 2017a). Apart from the phasing loop transfer phase, numerous uncertainties relating to LOI burns were also considered. Uncertainty requirement analyses for the orbit, attitude, and burn performance of the 1st LOI maneuver (Song et al. 2016a) were conducted. The effect of burn delays with the inclusion of position and velocity errors (Bae et al. 2016b, 2017b) were also analyzed. As part of the early phase contingency trajectory design and analysis activities, the required time of flight and associated delta-V magnitudes for each recovery maneuver to recover the KPLO mission trajectory were also analyzed, and focused on the direct recovery option (Song et al. 2017b).
For the successful operation of the KPLO, the FDS for the KPLO ground system (GS) was initiated in 2016. Song et al. (2016b) conducted the preliminary design of the FDS of the KPLO mission. The FDS for the KPLO mission will be developed to support all phases of the KPLO mission, including the launch (after separation), lunar transfer, LOA, commissioning, nominal operation, extended mission (if necessary), and end of mission phases. The system configuration of the KPLO FDS was also discussed, including details of the functionalities of each of the modules, with brief discussions on the overall architecture of the GS for the KPLO mission (Song et al. 2016b). As the KPLO FDS must be developed to not only guarantee the successful flight operation of the KPLO, but also to secure the core technologies related to planetary flight dynamics and navigation in Korea, the KPLO FDS adopted the concept of the dual simulation engine, and the associated system platform is under development. The KPLO FDS will be capable of simulating orbital dynamics during the flight operation utilizing both a commercial-off-the-shelf (COTS) simulation engine and the KARI simulation engine. The COTS simulation engine will be developed using the widely used STK and Orbit Determination Tool Kit (ODTK) by AGI, and the KARI simulation engine will be developed using previous KARI low earth orbit (LEO) and geostationary earth orbit (GEO) mission FDS development and operation data (Song et al. 2017c). The KARI has successfully launched more than ten Earth orbit satellites, including LEO and GEO, and successfully operated the FDS for each satellite. Although KARI has both the FDS development and operation data from LEO and GEO missions, preparing for successful operation of the KPLO FDS remains challenging in certain aspects. Therefore, the functional differences of an FDS between the LEO and GEO satellites and the KPLO mission was critically re-evaluated (Song et al. 2018). There are a number of functional modules nested inside the KPLO FDS to cover different functional roles, including system management, maneuver planning (MP), orbit determination, and fuel accounting. Song et al. (2018) reported new functions implemented into each module of the KPLO FDS that were not covered by the FDS for Earth missions (Song et al. 2018). The top level KPLO FDS functional architecture is shown in Fig. 4 and the top level dual simulation engine concept of the KPLO FDS is shown in Fig. 5.
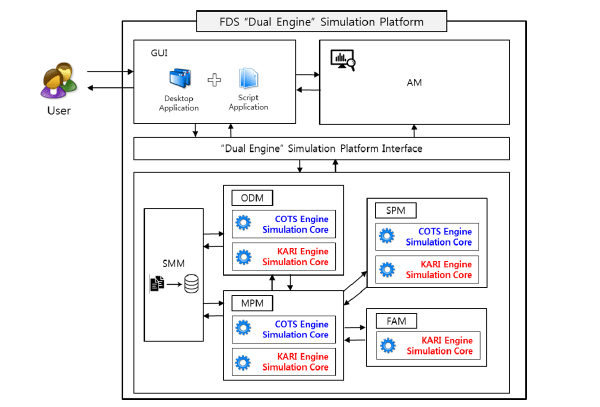
Apart from trajectory design and analysis with the inclusion of the MP discussed above, considerable effort has been invested regarding deep-space navigation and operation to ensure successful operation of the KPLO. Song et al. (2017a) analyzed the effect of applying different selenopotential models to the propagation of a lunar orbiting spacecraft by applying a current seleno-potential model, the GRAIL1500E model. From the viewpoint of successful KPLO navigation, numerous studies as well as analyses have been conducted considering the effect of measurement acquisition conditions and dynamic process noises on lunar orbiter OD performance, and determination and prediction simulation of KPLO mission orbits. (Kim et al. 2016a, b, 2017a, b, c, d, 2018a, b). Kim et al. (2016a) summarized LOI strategies of previous lunar missions and investigated associated OD requirements and actual performance for a preliminary analysis of the KPLO OD. An OD analysis for a lunar orbiter at 100 km altitude was conducted using various measurement acquisition conditions for a KPLO nominal mission (Kim et al. 2017a). Kim et al. (2017a) generated an orbit ephemeris of a lunar orbiter, which has a 100-kmaltitude lunar polar orbit, and the NASA orbit ephemeris for the Lunar Prospector was used for initial orbit information. Sequential ranges and Doppler measurements of a lunar orbiter by three DSN and one KDSA were simulated. Kim et al. (2017a) reported that stable and accurate OD performance was observed in measurement acquisition conditions with passes of greater than 30 min/per orbit. To verify the OD and orbit prediction strategy established for the KPLO mission, Kim et al. (2017c) analyzed expected OD and OP performance using actual LRO tracking data. Kim et al. (2018a) examined the influence of the lunar gravity model on the OD of a lunar orbiter operating at an altitude of 100 km in a lunar polar orbit. Kim et al. (2018a) reported that significant improvements in OD accuracy were observed by applying a GRAIL-based model. Applying a full order and degree gravity modeling was not always the optimal strategy because of the computational burden, and they concluded that GRAIL660B with a 70 × 70 degree and order was the most efficient strategy for mission preanalysis. In addition to these efforts, Kim et al. (2017e) presented an overview OD software based on an in-house code that was developed to partially support KPLO FDS. This software had three primary capabilities: celestial event prediction for spacecraft, orbit determination with DSN tracking data, and DSN tracking data simulation. Kim et al. (2017e) also reported validation and verification results. Lee et al. (2017b) demonstrated and showed validated results of the Kim et al. (2017e) software in a 100-km-altitude lunar orbit using actual Lunar Prospector tracking data. The modeling characteristics of the MP and OD functions of the KPLO mission are presented in Tables 1 and 2.
For the successful flight operation of KPLO, not only the design philosophy but also the performance of the FDS will be verified and validated in a number of steps. System architecture, interface, data management, operational flow design, including astrodynamic-algorithm development, will first be performed by KARI. Design results, including operation flow and performance, will be validated via technical consulting from Space Exploration Engineering, which is a U.S. corporation specializing in deep-space trajectory and mission design that supported more than 30 operational space missions. Space Exploration Engineering (SEE) headed the NASA Interstellar Boundary Explorer (IBEX) mission trajectory design in 2008 and also led the design and operation of cislunar trajectories for the NASA Lunar Atmosphere and Dust Environment Explorer (LADEE) in 2013. After successful development of the FDS, the second step of the performance validation of the FDS will be performed. During the operation of the KPLO, realtime performance will be validated and verified with NASA Johnson Space Center (JSC) including upcoming maneuver planning results, orbit determination results, as well as support of contin-gency trajectory designs with conjunction analysis.
4 PREPARING FUTURE MISSIONS: LUNAR LANDING, ASTEROIDS, AND BEYOND
After completion of the KPLO mission, Korean planetary exploration missions would be boosted. On the basis of the recently announced 3rd Basic Plan for Promotion of Space Development, Korea is now preparing Space Korea Vision 2040. It is expected that detailed action plans, aims, and scopes of Korean future planetary missions will be declared and announced by early 2019. From the KPLO missions, infrastructure technology for planetary missions could be secured and this could be a significant cornerstone to attempt diverse exploration missions to other planets with more advanced concepts. In addition, the successful establishment and operation of the KDSA during the KPLO mission will enhance deep-space navigation capabilities to more precisely target other planetary missions; these capabilities are essential to meet more stringent performance requirements. According to the 3rd Basic Plan for Promotion of Space Development, mission analysis and technical standard reviews for the moon landing mission will commence in 2019, and it also declares that asteroid landing and sample return missions will be conducted by the end of 2035. In fact, diverse concepts of technical assessments have already been conducted in order to prepare for the future of Korean planetary missions.
The current KPLO mission utilizes the concept of high thrust. Moreover, most of the relevant trajectory design and analysis studies to date have been conducted using the high thrust concept. However, in addition to this concept, the application of low thrust to planetary mission trajectory design and analysis have also been performed (Lee & Bang 2007, 2009a,b; Song et al. 2009b,c; No & Jeon 2010; Lee et al. 2012). Lee & Bang (2007) derived optimal low thrust trajectory solutions to design Earth–Moon transfer trajectories with a 2-dimensional problem. Song et al. (2009b) reported optimal Earth–Moon transfer trajectories using both constant and variable low thrust with more detailed flight dynamics, 3-dimensional problems, and 3rd body perturbations. No & Jeon (2010) reported an Earth–Moon transfer trajectory design using mixed impulses and continuous thrust. Song et al. (2009c) proposed a lunar cargo mission design strategy using variable low thrust by combining both analytical and numerical optimization methods. As reported in the Song et al. (2009c) study, de-termining initial estimates for spiral shaped transfer trajectories is a highly sensitive and timeconsuming job. Therefore, in order to address these problem and to obtain greater insight, Lee & Bang (2009b) examined behaviors of initial costate over the transfer time and initial radius of a circular orbit, which is critical when optimizing spiral shaped orbit transfer problems. Lee & Bang (2012) reported a new initial costate estimation method to solve the specific energy targeting problem defined in the Earth– Moon rotation frame. To study and analyze lunar lander trajectories, Park et al. (2008, 2009) performed minimum fuel landing trajectory analyses considering attitude variations during the landing phase. Cho et al. (2009, 2010) analyzed optimal lunar landing trajectories with knowledge of parking orbits before the descent phase, and Jeong et al. (2010) presented a precise planetary landing method with terrain-aided inertial navigation. Jo et al. (2010) designed and analyzed optimal descent/ascent trajectories for a lunar lander mission with segmented detailed sub-phases of the landing phase. Park & Rew (2018) proposed a lunar landing scenario of a robotic mission and derived an optimal landing trajectory considering the attitude angular velocity variations.
Apart from these efforts, diverse areas related to planetary missions have already been initiated to prepare for future planetary missions. These studies include a planetary rover mission (Kim et al. 2012; Eom et al. 2012, 2014), dynamic simulator development (Rew et al. 2010), conceptual asteroid mission trajectory design (Yoo et al. 2009; Song et al. 2010c; Song & Park 2015), and a planetary mission trajectory concept generation using small satellites (Song et al. 2015b, 2015c, 2017d, 2017e; Lee et al. 2017c). From these combined studies, it is believed that the cornerstone of the future Korean planetary missions, such as a lunar landing or asteroid sample mission, would be more achievable. In addition, future missions will build on these studies. Although sustained efforts are still essential to realize such challenging future planetary missions, Korea is now assuredly making progress, not only technologically but also regarding space politics, to realize the goal of future planetary exploration missions.
5 CONCLUDING REMARKS
Korean space technologies have grown rapidly since the first launch of Korea Multi-Purpose Satellite-1 (KOMPSAT-1) in 1999, and aspirations are rapidly expanding to encompass outer space exploration. Unlike earth orbiting missions, planetary missions are more demanding of well-rounded technological capabilities, specifically trajectory design and analysis and navigation abilities. To accommodate these essential components, numerous efforts to secure these basic technologies have been conducted by the Korean astronautical society. By performing conceptual Mars mission trajectory design and analysis during the early 2000s, Korea has secured a basic knowledge in the areas of planetary mission trajectory design and analysis. During the late 2000s and early 2010s, the target planet was switched to the Moon and continuous efforts have been devoted to prepare an actual lunar mission. After the funding for the first KPLO was initiated in 2016, detailed trajectory design and analysis studies were conducted with more concrete successful launch and operation plans of KPLO missions These plans included cooperation details and role assignments for lunar exploration technological cooperation between KARI and NASA. Among the various technological cooperation agreements between KARI and NASA, KARI is now focusing more on trajectory design, analysis, and operation activities, including joint efforts between KARI and NASA, to validate trajectory design and navigation performance. Completion of the KPLO mission could secure infrastructure technology for planetary mission trajectories, and this could be a significant cornerstone for Korean attempts for diverse exploration missions to other planets. In addition, deepspace navigation capabilities will also be secured by the successful establishment and operation of the KDSA. Apart from efforts devoted to the KPLO mission, diverse technical assessments have been already conducted in order to prepare for future Korean planetary missions, including low thrust trajectory transfers, lunar landing trajectories, rover mission preparation, dynamic simulator development, conceptual asteroid mission trajectory designs, and planetary mission trajectory concept generation using small satellites. However, sustained efforts are still essential to realize such challenging planetary exploration, and Korea is now in an era of changing and expanding areas of the national space program, and devoting its best efforts with confidence under limited funding and human resources. There is no doubt that past and current flight dynamics and navigation efforts devoted to planetary missions to date will enhance relevant technological capabilities in Korea, and be significant milestones in Korean space development history.